Colloquy Podcast: A Step Closer to Personalized Medicine
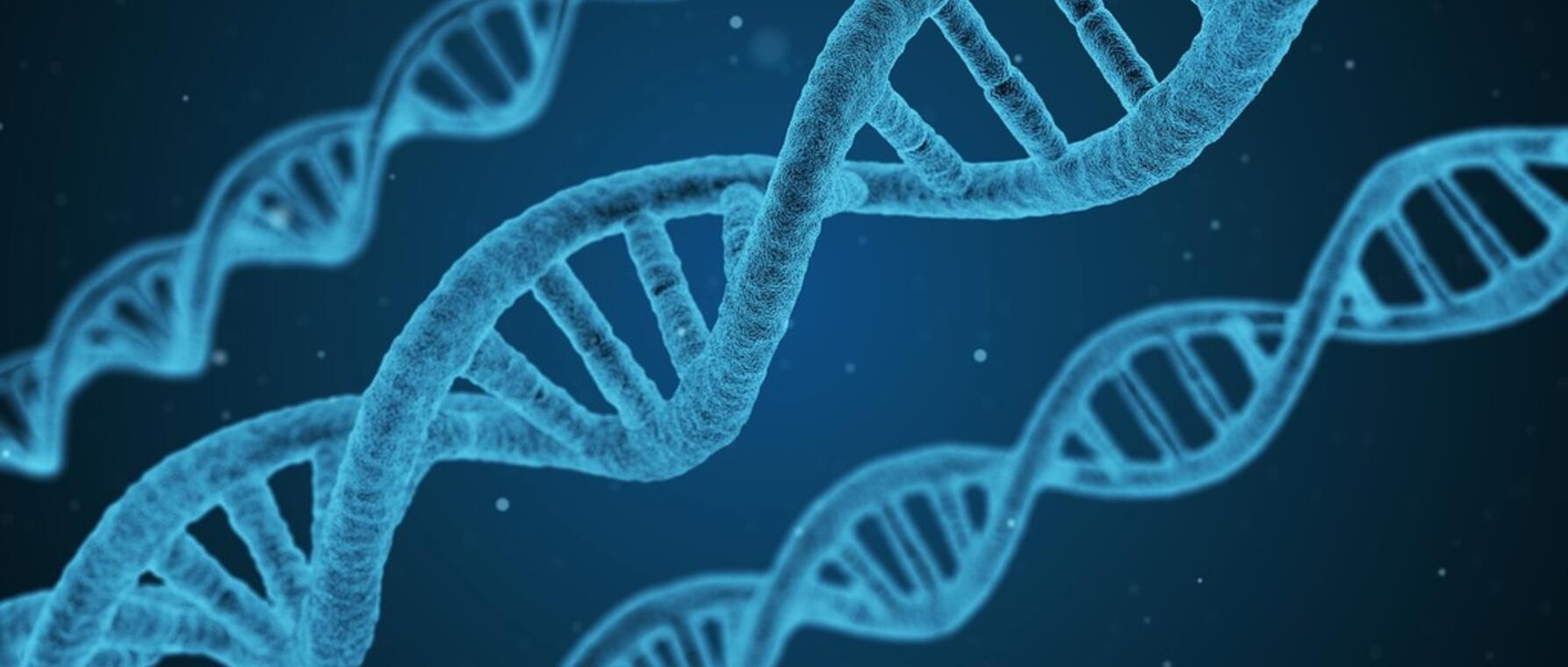
Research at Risk: Since World War II, universities have worked with the federal government to create an innovation ecosystem that has yielded life-changing progress. Now much of that work may be halted as funding is withdrawn. Find out more about the threats to medical, engineering, and scientific research, as well as how Harvard is fighting to preserve this work—and the University's core values.
Imagine your doctor could precisely predict your personal risk of disease, diagnose the cause of illness with pinpoint accuracy when it did occur, and develop an effective treatment plan with low side effects the first time, rather than through trial and error. That's the promise of personalized medicine. And it would be a revolution in healthcare.
At the heart of this vision is the notion that our genetic differences have a big impact on how each of us responds to disease and treatment. To realize a future of personalized medicine then, we need to understand and investigate just how genetic variations, including mutations, contribute to illness and respond to doctors' attempts to address it. But how can scientists do that efficiently with a human genome that spans about three billion base pairs of DNA across tens of thousands of genes?
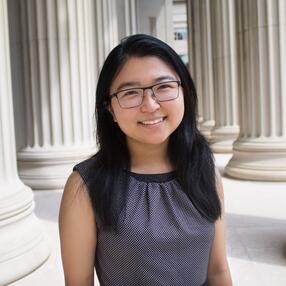
That's where the work of PhD student Dawn Chen comes in. A student in Harvard’s Department of Stem Cell and Regenerative Biology and the Systems, Synthetic, and Quantitative Biology Program, Chen was named a recipient of the 2025 Harold M. Weintraub Graduate Student Award for Outstanding Achievement and Exceptional Research in the Biological Sciences, presented by Seattle's Fred Hutch Cancer Center.
With her colleagues in the lab of Harvard professor Fei Chen, Dawn Chen is developing an innovative gene-editing tool known as helicase-assisted continuous editing, or HACE. A breakthrough in genetic engineering, supported in part by funds from the National Institutes of Health, HACE makes edits to specific genes, allowing researchers to investigate how genetic variations contribute to disease. The technique could lead to the identification of specific mutations that influence the effectiveness of drugs and therapies for illnesses like cancer.
(This transcript was edited for clarity and correctness.)
So just to set the table, can you give a brief primer on gene editing, what it is, how it works, and why it's such an important tool for scientists today?
So gene editing really refers to making changes to your DNA. All of us have DNA inside of all of our cells. And that is the instructions that tell us how to become a person really. It's a little bit like our biography or a book that tells us how we are as people.
There are a lot of people who are born with different genetic diseases. For example, they might have a typo in their book of life. And as a result, they might have different diseases. Like you might have heard of progeria or cystic fibrosis, or even sickle cell disease. These are all because they have certain genetic mutations inside them. And as a result, they might be very sick. And as scientists, we want to correct those mutations and make the person healthy again, which is why we might want to edit that person's genome or DNA.
And the process of gene editing really requires you to, first, figure out where it is on the genome that you need to make changes to. And then afterwards, you can go in and just—ideally, you can use some sort of correction tape where you just wipe out the change and replace it with something that is the correct gene at the location.
And in terms of humans, there are maybe a few different ways of doing that. And yeah, so historically, there have been many, many decades of development in this area. Like, historically, there have been TALENs (Transcription Activator-Like Effector Nucleases) or zinc fingers [both types of pre-CRISPR gene-editing techniques]. And these days, we have a lot of gene editing using CRISPR-Cas9.
Many of our listeners, I'm guessing, have heard of CRISPR. But they may not fully understand what it does. Could you give us a brief explanation of the technology and what its role is in gene editing?
CRISPR is actually a bacterial immune system that it uses to defend against all these kinds of hosts of pathogens. Scientists took this CRISPR tool and made it such that we can use it to edit DNA at a precise location.
To do CRISPR editing, you really need two components. One is that you need a Cas9 protein, which really is a pair of scissors that can cut DNA at a location. And how you tell the Cas9 protein where to cut is, you have this guide RNA that tells the Cas9 protein where to go. So then, the guide RNA will go in and find the location that needs to be cut. And then, the Cas9 enzyme, which is this protein, actually goes in and cuts the protein at that location. So that is how CRISPR-Cas9 really works.
And actually, a lot of people have developed different ways of using this tool to make edits. Because even though the “vanilla” Cas9 really is just an enzyme that cuts DNA, you can fuse it to another protein, like, for example, one that makes base changes, or can insert or delete DNA. And then, you can make different kinds of gene-editing tools.
So given the state of gene editing, what's the problem that you were working on with your research?
I was actually trying to figure out ways to do mutagenesis in mammalian cells. And what that really means is to make mutations in the DNA of mammalian cells at scale, but in a targeted way. And the reason we want to do that is, historically, there has been a human genome project that has identified or sequenced the entire human genome. And now, we know where every base is.
And people have also done a lot of what you call genome-wide association studies, or GWAS studies, which tell people what are the different genetic mutations that different people have. And by just observing maybe a few million people at scale and looking at what kind of genes they have, and also what kind of disease they have, you can make some predictions or inferences about which of these mutations in their genes are actually important.
But really, this is just an observational study. And you need ways to validate it in the lab. And how you can do it is, usually scientists will maybe take some cells, grow them in a dish. And then, you can install these mutations that you observe in patients and see what kind of changes they lead to.
For example, if I observe that you have a few mutations, I want to be able to know for sure which one of them is actually important for the disease that you're having. I can only guess, right? So we will need to actually test them in the lab.
But the process of making these mutations is not as trivial as you think. People usually would maybe synthesize a few pieces of DNA outside the cell and then put them inside cells. But that actually is not great because a lot of times, you can't just put something created outside a cell that is artificial inside a cell and assume it will work in a cell in the same way. And so, we really wanted to make a tool that can make changes in human cells at a targeted location, but also over large ranges, so that we can really understand the function of individual genes better.
Now, we're talking about helicase-assisted continuous editing (HACE). So, how does it work? And how does it work with CRISPR-Cas9?
HACE is the first way to make scalable mutations at a targeted location inside living mammalian cells. And you can also make mutations over time. So it’s a way of editing, but it's also a way of encoding, like simulating evolution in some sense. Because evolution really is a process of making mutations over time.
And so what HACE really does is it has two components: first is a CRISPR-Cas9, which is a set of instructions that tells the cell where you want the edit to be. For example, if I were to study a gene of interest, like a cancer-associated gene, maybe I would want to just target that location of interest. And then next, after the Cas9 actually finds the location, then we have this secondary protein that we engineered to fuse a helicase to an enzyme that makes base edits.
A helicase is a little bit like a protein that unwinds DNA. So it's a little bit like a zipper on your jacket. The helicase can move along the DNA. And DNA really is a little bit like a zipper because it has two strands. And so the helicase will unzip the DNA. And when we fuse this helicase to a base-editing enzyme, the helicase scans along this target location, then the editing enzyme, which is the aid, will also make mutations as the helicase scans along this stretch of DNA.
So the helicase kind of unzips the DNA, and then this enzyme trails along with it and makes mutations to the DNA. And we can target it with CRISPR-Cas9 because the helicase is actually recruited to the location where the Cas9 enzyme is.
So the Cas9 is kind of like a GPS?
Yes, that's right.
I know that you've done a lot of work with cancer. You got the Weintraub Award from the Fred Hutch Cancer Center. Can you talk about how you use this technology to understand cancer better?
Yeah. We have actually applied our tool to understand two different systems. The first is the MEK1 kinase system. MEK1 is an enzyme that is a kinase that is very frequently mutated in different cancers. And generally, let's say if you have skin cancer, a lot of times, if you were to go to the doctor, the doctor might give you a drug that inhibits the MEK1 protein as your treatment.
But unfortunately, a lot of times, people develop resistance to MEK1 kinase inhibitors or drugs over time. And sometimes, we just wanted to really understand what are some of the mutations that can lead to drug resistance in the MEK1 gene. This would be really helpful, for example, if you were to go and see the doctor, and you realized that you were on this drug, but it doesn't work anymore. The doctor can look at you and just look at your DNA and be like, hey, actually, I know what the mutation is that's leading to your resistance.
Or, if you can really understand how this protein becomes resistant over time, then we can develop better drugs to prevent these mutations or resistance mechanisms from happening beforehand. And so, we were able to use our tool to identify different mutations in MEK1 that lead to drug resistance.
And the other thing that I studied was this other protein that is very close to my heart, which is called SF3B1, which functions as a kind of splicing protein. It is also very frequently dysregulated in different kinds of cancers. It’s a very interesting protein because alternative splicing is something that I study very deeply as a process. And this process is also very dysregulated in many kinds of blood cancers. And that results in your cells making the wrong proteins that have—yeah, so it sort of affects this global process.
And in this case, we really wanted to see what the mutations are that actually lead to this protein making wrong proteins over time. And we were able to find mutations in this SF3B1 protein, that are actually the functional ones, that lead to it being—going rogue kind of thing.
There's a lot of talk about personalized medicine or customized medicine. What does that mean to you? And how do you see your research, if at all, contributing to making that a reality?
I really want to understand all three billion bases in the human genome and what every single base does. Right? Because if we can really understand what every single base does, then that means that we can really make different therapies. Because if you want to control biology, you first have to understand it. And so we are at a stage where we have understood enough that we can make this CRISPR-Cas9 tool to really treat diseases. But there's really a lot more biology that we don't understand that we want to know of.
And I think: if we really can understand all the different kinds of biology of the cell, then we can really make different kinds of therapies that will just work. And I think a lot about this in the context of different rare diseases. There are a lot of people who suffer from different kinds of rare diseases. You can see that they are definitely sick because maybe they are children who have developmental delays or other kinds of effects. But we are not sure what really is wrong with them. And so I think it would be really cool to just be able to understand the individual impacts of different mutations and changes in our genome. And then, the next step is, of course, if we can also make better gene-editing tools, then we can really be able to, not just understand it, but really correct its mutations.
You've just talked about wanting to better understand the biology of the cell, that there's a lot that we've learned about genes and gene editing and DNA. But there's still a lot that we don't know. So I'm wondering: what are some of the challenges that you face in the research that you're doing and what you're trying to understand?
Something that we really want to be able to do is not just introduce these mutations to these cells once, but do it over a long period of time to be able to evolve and engineer new cells with new functions. And we have actually already been able to do this in bacteria a little bit, and also in yeast. For example, you can make different enzymes that are in your detergent that work very well. Or we can also be able to use bacteria to evolve different proteins. Like for example, enzymes that can degrade plastics. And those, we can all evolve in bacteria.
But there are a lot of different human proteins that we can't just evolve in bacteria. And in these cases, we will have to evolve it in human cell systems. And so, I kind of imagine that there could be a day where we can, not just make individual molecules like enzymes or proteins, but we can really evolve cells themselves to do certain functions. Like, maybe we can evolve cells that can just go into your body and just know to go and kill all the cancer cells because it knows some signal. And that would be much better than any of the drugs that are available right now. And this is actually the future that I hope for. Yeah.
That's a great segue to my last question, which is, what does the future look like for gene-editing technologies like CRISPR?
In terms of my research, I'm actually really interested in cell-type specific targeting, which is how you can express something, for example, how can you express a drug in the brain but not in the liver? Or how can you express a drug just in cancer cells but not in non-cancer cells? This is something that I'm actively working on right now to really harness in very clever ways, different biological mechanisms that already exist inside the cells to do this kind of research.
I think in terms of the field of gene editing, I'm really looking forward to seeing all the different—I mean, there has already been a lot of progress in this field. And we see that there have already been FDA-approved therapies using CRISPR. So that's, like, super, super exciting.
At the same time, I also think that one of the biggest challenges with these tools is delivery. It's still not trivial to get these CRISPR enzymes or different kinds of gene-editing machinery inside human cells, especially if you were to give it to a person as a therapy treatment. And so, a lot of people are working on different delivery challenges. And I think that once that becomes better, then we can really have a pipeline of one, yes, we understand the biology, and two, we can make something that can correct it. And three, we can really deliver it to the patient, leading to a good outcome.
Get the Latest Updates
Join Our Newsletter
Subscribe to Colloquy Podcast
Simplecast